“Electric bacteria” pass electrons through cell walls to solid minerals outside the cell. El-Naggar studies how and why this amazing process happens.
September 22, 2020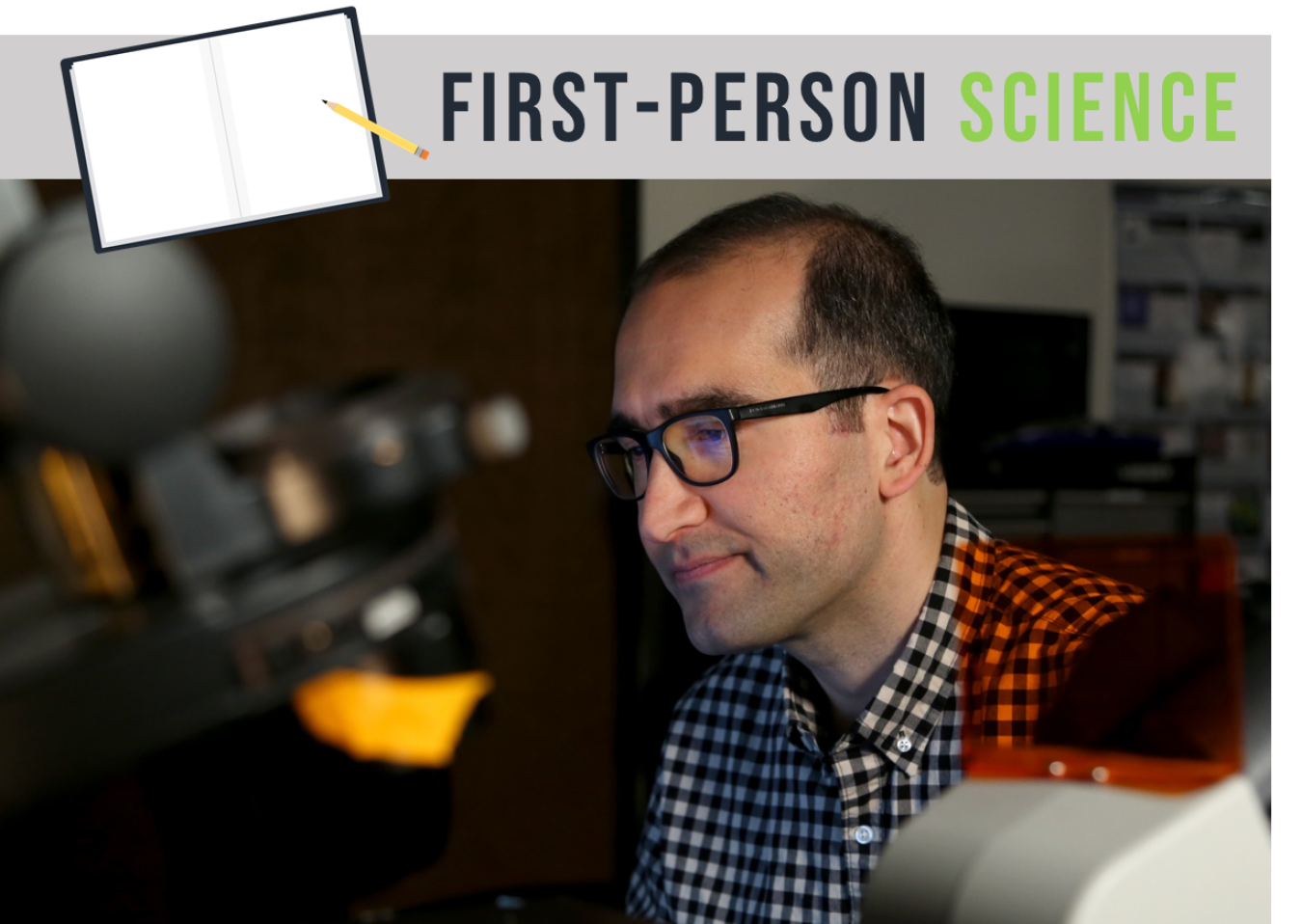
In the First-Person Science series, scientists describe how they made significant discoveries over years of research. Moh El-Naggar is a professor in physics, astronomy, and chemistry at the University of Southern California Dornsife. He was also selected for the Presidential Early Career Award for Scientists and Engineers.
What really drives me are questions of limits. As a physicist working in biology, I wonder, ‘What’s the maximum thing possible that biology can do? What is the most you can get out of this system? How complex can this environment be and biology is still able to work?’ I think the best way to learn about the physics of anything, including biology, is to ask questions about limits.
In my own field, I’ve seen how asking these questions can lead to answers we would have never anticipated. I study bacteria that the popular jargon refers to as “electric bacteria.” The more specific terminology to use is metal-reducing bacteria. All organisms store and use energy through chemical reactions that move electrons. Many organisms move electrons from food and then discharge them into chemicals within their cells. For organisms that use oxygen, the last step in the complex process of converting energy is having an oxygen molecule accept electrons to become water. Metal-reducing bacteria are different. Instead of having a chemical reaction with a molecule inside the cell, their last chemical reaction passes electrons to solid minerals outside the cell. So for that chemical reaction to happen, they have to figure out a way to move this electron through the cell wall to get it outside the cell and beyond. Sometimes, these bacteria move electrons hundreds of times further than the bacteria’s diameter. That’s something only microbes can do!
When scientists first discovered these bacteria in the 1980s, this ability baffled them. Because the membranes that surround the cell are made of fatty molecules, they act as insulators. Electrons shouldn’t be able to move across them.
My field of electromicrobiology seeks to understand how microbes transfer electrons outside the cells and between each other. It can help us understand how life evolved and possibly develop technologies that harness these processes.
This field is still at its beginning. Right now, we’re in the Galvani frog moment. Imagine you’re Italian scientist Luigi Galvani in 1780. You’re working on a frog and start poking electrodes into it. The frog starts moving! Fast-forward a couple hundred years and all of a sudden you’re talking about neuroscience. But you can imagine how exciting and ‘weird’ it was when the phenomenon was first reported. For the kind of work that we do, we’re still in that Galvani frog moment. As we gain understanding, there are also interesting applications that might actually work.
“Like a Sci-Fi Thing”: 2006-2008
While I’m usually more interested in basic questions than applications, one of those applications was what drew me into the field. Although my PhD was not related to biology, towards the end of it, I got interested in the amazing things microbes can do. As I was looking for a postdoc position, I learned about microbial fuel cells. Instead of traditional fuel cells that use metal catalysts to convert energy, the catalyst in these happens to be a living, breathing organism. The innards of it are actually alive. It was like a sci-fi thing to me.
I started looking for a job I was utterly unqualified for. I cold-called Ken Nealson at the University of Southern California (USC), one of the founders of this field. He said, “Why don’t you just come down and let’s chat this afternoon?” Within 15 or 20 minutes of our conversation, he offered me a job. To be honest, I was taken aback.
My timing was essentially perfect. It’s only been in the last 15 years that we’ve come to appreciate how these bacteria move electrons at the molecular level. I was starting in an area at a time when a lot of the advances were happening.
Asking the Basic Questions: 2009-2010
Physics is powerful when applied to biology. Physicists search for organizational principles and mechanisms before going into the nitty-gritty details of a system. When somebody gives you an observation or proposes a new mechanism, physicists can come up with ways of rationalizing whether it’s possible or not. That line of thinking leads you to the next question and the question after that.
In many cases, we couldn’t investigate those questions until we developed the tools to do so. This is not a well-developed field where the standard techniques are all worked out. We spend a lot of our time trying to figure out how to even do the measurement.
Back in 2009, early in my time at USC, we were investigating bacterial nanowires. These filaments string out of the cell and are only as long as a fraction of the width of your hair. While other researchers had inferred that these bacterial wires transferred electrons, we wanted to use physics tools to measure the transfer rate.
At the Molecular Foundry, an Office of Science user facility at Lawrence Berkeley National Laboratory, we went through a lot of trial and error. The Molecular Foundry has fantastic pieces of instrumentation where you can go in and build contacts to really, really, tiny structures, like between the electrodes and nanowires. I commuted to Berkeley once a week, flying out of Los Angeles early in the morning and coming back that night or the next day. I spent a lot of time in a cleanroom wearing what researchers call a bunny suit, building one contact after another. Sometimes they’d get broken, sometimes they didn’t connect, sometimes the samples were no good. But one evening, late at night and by myself, I successfully put all of the pieces together. I made contacts at both ends and then as I applied voltage to them, watched the electricity flow. I remember walking back down the hill from Berkeley Lab that night with an extra spring in my step.
It was a great starting experiment in the sense that it taught us that electron transfer in these nanowires was possible. We found that these bacterial nanowires transferred current at a rate similar to silicon nanowires. This experiment also taught us about the molecules that were involved, called cytochromes. By making mutant bacteria that were missing the cytochrome genes, we found that cells that didn’t have these particular molecules grew filaments that couldn’t move the electrons.
Looking back, I still think it’s amazing work. But I also think, ‘Man, did I learn so many better ways of doing measurements that respect the integrity of biology.’ We were really using these tools that were not designed to work with living samples.
Since then, we’ve invented new techniques to work with cells while they’re actually alive. Often, we spend a few years building up that capability from scratch. In this academic world where you have pressure to come up with publishable studies every year, it takes bravery from my students to jump into a project where they have to build things from scratch to get an outcome years later.
From Molecules to Biofilms and Back: 2011-2018
Once we did that experiment, we wanted to connect the dots between molecules and the activity of whole cells, and even groups of cells.
In 2014, we started by looking at the mechanism of how electrons move in the individual molecules. From a combination of theoretical modeling and single molecule measurements, we learned that the electrons move in a multi-step process. The process is like water in a bucket brigade, with the electrons hopping between metal sites inside each cytochrome molecule. Based on this, we set some limits on how much electron current can flow in each molecule.
Knowing what a single molecule can do, we then turned our attention to cells in 2015. How much current does each cell need to survive? There was no off-the-shelf solution to make this kind of measurement. We pulled pieces from optics, electrochemistry, and microfabrication to do the measurement. By trapping individual bacteria with lasers and landing them on tiny electrodes, we saw that each cell transmitted a tiny current. That’s more than a trillion times smaller than the current running in your energy-efficient light bulb at home. There’s so much that a living organism does with this little power budget. It turns out that bacteria are the ultimate energy conversion machines. We have so much to learn from the oldest inhabitants of the planet.
We could now connect the dots between molecular and cellular measurements. Each cell needs as little as a handful of cytochromes to transmit its total current.
Moving on from single cells, in 2018, we started looking at how the electrons move in whole biofilms of metal-reducing bacteria. These thin layers of microorganisms live on surfaces like rocks as a group. By measuring biofilms covering gaps between microelectrodes, we eventually found out that the cells can collectively act like a big organism, moving electrons from one cell to the next. Once again cytochromes, the all-important electron transfer proteins, were key to transmitting the electrons across cells.
At the same time, we also went back to take a closer look at the bacterial nanowires. Analyzing what they were made of could help us better understand how they might be transmitting electrons. We had been pursuing a hypothesis that hair-like protein appendages called pili were somehow involved, along with cytochromes, in forming these wires. But along the way, we realized we had the completely wrong idea about their nature. In the organism we’re studying, the nanowires are made of membrane tubes studded with cytochromes. That was a fairly big surprise. We walked into it thinking one thing and the data led us in this completely different direction. That discovery helped us identify a second possible mechanism for how the membranes of wires and cell surfaces pass along electrons. Careful imaging and modeling showed that bacteria might use the way electron transfer proteins float around on the membranes and bump into each other to move electrons efficiently.
A lot of times, scientists just go down a rabbit hole that turns out not to be the right answer. But we know so little that every time we went down one of these rabbit holes, we ended up learning what wasn’t the answer, which was almost as useful as the answer itself. Attacking it one step at a time from molecules to cells to biofilms, and now back to looking at the movement of molecules, we now have a clearer picture of how it works than we did 15 years ago.
Going Beyond Natural Capabilities: 2019-Now
We’re now getting to the point where we understand the natural processes well enough that we could apply them to technology. There have been incredible success stories in the last few years where people are deploying bacterial fuel cells for wastewater treatment, which usually requires a lot of energy to accomplish. Instead, these bacterial fuel cells are producing energy in the process. That’s something that went from being a curiosity perhaps 20 years ago to a technology that’s now being sold.
Lately, I’ve been looking at these bacteria and thinking of them in terms of developing electronics based on living cells. Biology has certain functions, like sensing and processing information, that it really excels at. I think now we might be in a place where we can ask questions about designing new functions that can even go beyond what nature is capable of.
My field has changed radically since I first read about that bacterial fuel cell. Two decades ago, when people said that electrons move long distances in biology, they meant a couple of nanometers. Since then, because of our discoveries around electron transfer in bacteria, we’ve jumped up to the micron scale and then the centimeter scale. If somebody had told me this fact 10 years ago, I would have put on a skeptical, grouchy physics face. I would have told you that’s not possible. But by walking in with a super open mind, we’ve found that these organisms exist and what they can do. I think there’s a whole lot of science that benefits from having an open, blue sky mind about what is possible.
The Office of Science is the single largest supporter of basic research in the physical sciences in the United States and is working to address some of the most pressing challenges of our time. For more information please visit https://www.energy.gov/science.
Shannon Brescher Shea
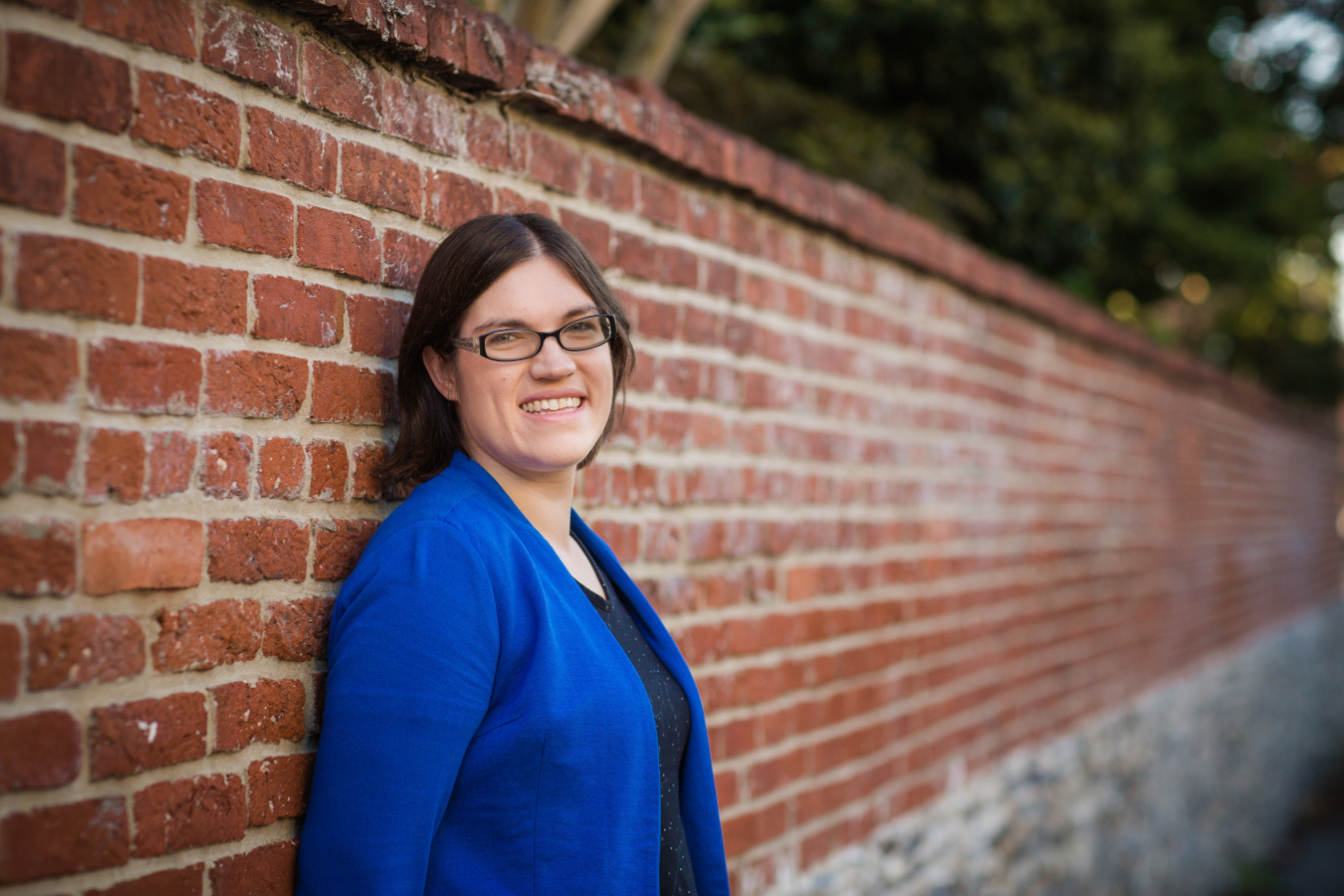
Shannon Brescher Shea (shannon.shea@science.doe.gov) is the social media manager and senior writer/editor in the Office of Science’s Office of Communications and Public Affairs. She writes and curates content for the Office of Science’s Twitter and LinkedIn accounts as well as contributes to the Department of Energy’s overall social media accounts. In addition, she writes and edits feature stories covering the Office of Science’s discovery research and manages the Science Public Outreach Community (SPOC). Previously, she was a communications specialist in the Vehicle Technologies Office in the Office of Energy Efficiency and Renewable Energy. She began at the Energy Department in 2008 as a Presidential Management Fellow. In her free time, she enjoys bicycling, gardening, writing, volunteering, and parenting two awesome kids.